Measuring and controlling the pH
Measuring and controlling pH is a very important aspect of bioprocesses, as changes in the pH can significantly alter growth conditions – usually with major consequences. Culture media commonly include buffers, i.e., substances that mitigate overly sudden changes to pH caused by the addition of an acid or base. Because an acid dripping into the culture medium can damage many cell lines, scientists often carefully enrich the gas mixture used in the cell culture with CO2 rather than adding a liquid acid. The gas then dissolves in the culture medium, allowing the carbon dioxide to influence the pH in combination with a buffer.
Typical pH ranges depending on the cultivated organism:
Organism
| pH |
Bacteria, Yeast, Fungi | 4.5 – 7.0 |
Mammalian cells | 6.7 – 7.4 |
Insect cells | 6.2 – 6.9 |
Plant cells | 5.5 |
For measuring pH during the bioprocess, each bioreactor is equipped with a pH sensor known as a combination electrode for pH. The bioreactor can correct any deviations in the pH; for this purpose, an acid and/or an alkaline solution is made available and connected to the culture vessel via tubes and pumps. The concentration of the acid and base must be skillfully selected for this to work – if it is too high, the drops of concentrated acid or base may damage the microorganisms and cell cultures before they are distributed in the bioreactor. If, on the other hand, the concentration is too low, operators will have to add more acid or base, unnecessarily diluting the culture medium.
Adding nutrients
During a bioprocess, microorganisms usually consume a wide array of nutrients. The basic composition of a nutrient medium usually consists of water, a usable energy source for the organism (e.g. glucose), as well as the nutrients it needs (carbon, nitrogen, and phosphorus), salts and trace elements. Depending on the organism, other compounds are necessary that cannot be synthesized by yourself (vitamins, essential amino acids, etc.).
Depending on the bioprocess strategy these nutrients are either all made available at the beginning of a bioprocess (batch bioprocess) or added over time such as in a fed-batch or in a continuous process.
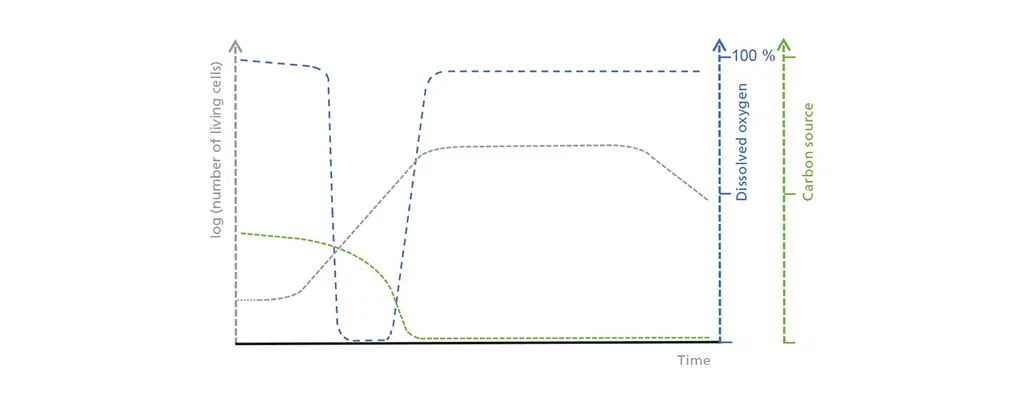
Schematic illustration of the correlations between living cell concentration, dissolved oxygen, and the limiting carbon source in batch operation. In the initial lag phase, the living cell count only increases slowly, which leads to a moderate but steady uptake of the carbon source. Oxygen consumption increases during the exponential growth phase until it exceeds possible oxygen input. Once the carbon source is depleted, the stationary phase starts and is followed by a dead phase, during which the living cell count drastically decreases.
If you’d like to find out more about the different feeding strategies have a look at our blog-post: The difference between batch, fed-batch and continuous processes.
Gassing
During the bioprocess, the bioreactor feeds a sterile gas mixture such as air into the culture medium. Constant stirring not only distributes the nutrients – it also reduces the size of the gas bubbles that arise in the culture vessel, thus efficiently releasing oxygen into the nutrient solution. This is important, because microorganisms and cell cultures can only absorb the oxygen that has been dissolved in the nutrient solution.
Oxygen demands vary: aerobic bacteria need oxygen, whereas others prefer gas mixtures such as synthesis gas (“syngas”). Anaerobic organisms, however, can do without gassing entirely, feeding only on inorganic and organic substances from the culture medium such as nitrate or fumarate.
Unlike microorganisms, cell cultures are gassed with more than just air – the oxygen content of the gas mixture can also be influenced using pure nitrogen and pure oxygen. The exact composition depends on the cell culture application. In order to keep the gas atmosphere constant, the bioreactor needs precise control systems.
At the beginning of the bioprocess, for example, a culture often needs less oxygen – and thus a smaller gas feed – since growth is still progressing slowly. Later, however, faster growth requires much more oxygen. In addition to ensuring a constant supply of the desired gas or gas mixture, the bioreactor also delivers the right amount of gas at the right time. To do this, the bioreactor has gas ports connected to pressurized air from the building, a compressor, or a gas cylinder.
The gassing rate is usually measured in liters per minute. In order to have a generic parameter applicable to various bioreactors, the rate is also frequently indicated as the specific gassing rate, which refers to multiples of the working volume (vessel volumes per minute, vvm) and is expressed as min–1. A typical value for microbial bioprocesses is 1 to 1.5 times the working volume per minute. For a bioreactor with 4 L working volume, the maximum gassing rate would therefore be 4 L * 2 L L–1 min–1 = 8 L min–1. In cell cultures, by contrast, the maximum rate is often 10% to 15% of the working volume per minute as a way of keeping gas bubbles small and thus preventing foam formation and damage to cells from bursting bubbles.
Changing the gas rate, however, is not the only way of controlling the efficiency with which the bioreactor delivers oxygen and other gases to the culture medium. The greater the surface area of the total number of all gas bubbles in the bioreactor – i.e., the more finely distributed the gas bubbles themselves – the more efficiently oxygen will be transferred from the gas to the liquid phase. This means, for example, that increasing the stirring speed can improve oxygenation for microorganisms, since the stirrer makes the gas bubbles even smaller and thus increases the total surface area of all gas bubbles in the bioreactor.
Precise regulation of pO2 – and thus precise control of the gassing rate and the gas composition – is very important since, normally, the pO2 should not be the growth-inhibiting factor for the culture. If insufficiently controlled, however, the pO2 does become the limiting factor.
Since the gas feed to the bioreactor is usually dry, moisture from the bioreactor can be collected in the exit stream during gassing. At a high gassing rate, not only would the fill level drop, but the moisture would also block the exhaust filter, preventing proper venting from taking place and allowing pressure to build up. To avoid this effect, bioreactors are equipped with an efficient exhaust cooler where the moisture in the exhaust condenses and can drip back into the bioreactor before it reaches the exhaust filter.
Measuring and controlling the pressure
The higher the pressure in the vessel, the more oxygen is dissolved. Culture vessels made of glass are frequently only approved for a pressure of up to 0.5 bar, which is not even half the pressure of a moderately filled bicycle tire. At a higher operating pressure, slightly damaged culture vessels made of glass can burst, which is a safety risk. That is why you should always ensure a free, non-pressurized exhaust line from the bioreactor by keeping the exhaust filter dry and regularly replacing it – in the process, you will also be ensuring the integrity of the culture vessel, of course. Unlike glass culture vessels, stainless steel bioreactors are designed for higher pressures and, even in their standard configuration, are suitable for pressures up to 2 bar (a well-filled bicycle tire). Plus, systems like these are often equipped with a pressure control mechanism based on a pressure sensor in the bioreactor and a proportional valve in the exhaust line. Not only can these measure the pressure in the bioreactor – they can actively control it as well.
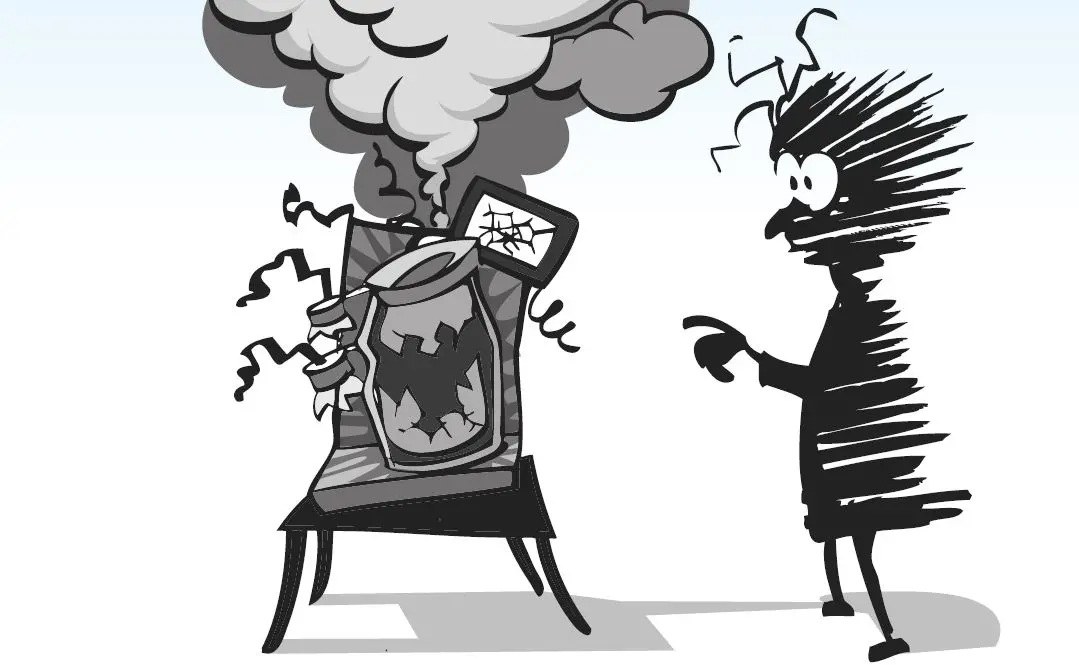
Preventing foam formation
Outside of bathtubs and beer glasses, foam is a rather unpopular side effect, especially in bioreactors. Foam forms at the interface between the liquid and gas phase in the culture vessel and can quickly find its way up under the top plate. In the worst-case scenario, it then blocks the exhaust filter, which in turn blocks the flow of gas. Most bioreactors are therefore equipped with a system for combatting foam formation. Mechanical foam breakers in the headspace are reserved for rather large stainless steel bioreactors, while antifoam control systems based on chemical agents (such as PPG, Struktol, or silicon-based defoamers) can be found in smaller bioreactors.
A typical antifoam control system consists of a sensor installed at a specific height in the culture vessel. If the foam height reaches the sensor, an antifoam agent is pumped from a reservoir into the culture vessel. These antifoam agents are active at the liquid-gas interface and increase the tendency of the foam bubbles to collapse. In particularly stubborn cases where the foam does not immediately dissolve, repeat the procedure after a preset time (a “shot & delay” strategy). Caution is advised when using an antifoam agent – if you dispense even slightly more than necessary, it can lie like a second skin on the surface of the liquid, which hinders the gas exchange. Antifoam agents also counteract efficient oxygen transfer, because the change in surface tension promotes the collapse of gas bubbles in the bioreactor, thus reducing the surface area available for gas exchange. The selection of the appropriate agent also depends on the bioprocess in question, because bacteria and cells react differently to certain chemicals.
Bonus: The benefits of using a SCADA software
Nowadays, the results generated in a bioreactor should be collected and evaluated as centrally as possible, as this is the only way of effectively implementing modern, big data algorithms in order to generate more information and to better understand how a process works. That is the job of the SCADA software. In the first step, all data from the bioreactor can be read out – with no major input on the part of the user – and stored centrally in order to evaluate it on its own or compare it with other batch data. This quickly triggers ideas for new experiments and possibly even complex batch strategies. A professional SCADA software lets you plan these easily and then control the bioreactor, which will ideally be a fully automated process. In addition, the SCADA software also integrates several components in the bioreactor environment. These include tools for process optimization using the design-of-experiment (DoE) technique or powerful software sensors, which can be used simultaneously to compute additional information directly from the batch process parameters and even to regulate those parameters. For example, the respiratory quotient (RQ) can be used to obtain an estimate of metabolic activity by means of the ratio of excreted carbon dioxide to absorbed oxygen.
There are so many possibilities – especially when the bioreactor and SCADA software are perfectly matched – that we could not possibly describe them all. If you are interested in learning more about all a modern SCADA software has to offer have look at the eve® bioprocess platform software.